Genome Editing & CRISPR
Genome (or gene) editing is an area of research that seeks to modify the DNA sequences of living organisms to, amongst other things, improve understanding of gene function and develop therapies against genetic and acquired diseases. Genome editing is a powerful tool that can be used to correct, introduce, or delete almost any DNA sequence into numerous cells and various organisms. Genome editing can be performed in vitro or in vivo by delivering the editing mechanism in situ through highly targeted genomic alterations.
Many current genome editing research has concentrated on viral infections, cardiovascular diseases, metabolic disorders, immunodeficiencies, hemophilia, muscular dystrophy/atrophy, and for the development of T cell-based immunotherapies. Some advances in genome editing have even surpassed preclinical research and are undergoing phase I/II clinical trials.
Biochemically, genome editing exploits the process of natural DNA restoration. When DNA within a gene breaks, subsequent nuclease-induced double strand breaks (DSBs) form. When a DSB is detected the innate repair mechanism of the body, known as recombination, will mend the DNA by stitching the break back together. Such recombination is most commonly done by one of the two major mechanisms; homology-directed repair (HDR) and nonhomologous end-joining (NHEJ).
Many current genome editing research has concentrated on viral infections, cardiovascular diseases, metabolic disorders, immunodeficiencies, hemophilia, muscular dystrophy/atrophy, and for the development of T cell-based immunotherapies. Some advances in genome editing have even surpassed preclinical research and are undergoing phase I/II clinical trials.
Biochemically, genome editing exploits the process of natural DNA restoration. When DNA within a gene breaks, subsequent nuclease-induced double strand breaks (DSBs) form. When a DSB is detected the innate repair mechanism of the body, known as recombination, will mend the DNA by stitching the break back together. Such recombination is most commonly done by one of the two major mechanisms; homology-directed repair (HDR) and nonhomologous end-joining (NHEJ).
Mechanisms
Homology-Directed Repair (HDR)
Homology-Directed Repair (HDR) is a process where DSBs are repaired by homologous recombination (HR) using a donor DNA template. HR is recognized as the earliest form of genome editing, developed in the late 1970s, after scientists followed the observation that yeast carry out HR naturally.
In HR, genetic information is exchanged (recombined) between similar (homologous) strands of DNA. In HDR, a DNA template is generated from within the cell during late S or G2 phase of the cell cycle, when sister chromatids are readily available prior to mitotic completion, and then isolated. The exogenous repair template is delivered into a cell commonly in the form of a synthetic, single string (ss) DNA donor oligonucleotide or plasmid to stimulate a precise change in the genome. In the presence of a HDR template, gene corrections or additions induce a DSB at the targeted locus. Once a DSB is achieved, HDR reconstructs the separated DNA using the exogenous DNA template.
Unfortunately HR, and therefore HDR, comes with several highly limiting traits. HDR is extremely inefficient in most cell types, specifically mammalian cells, which are commonly used as model organisms. Additionally DNA is double stranded and because HDR only manipulates a single strand of the DNA, this technique may lead to inherent inaccuracies.
HDR exhibits a high rate of error as the injected DNA fragments can potentially insert into an unintended part of the genome, leading to off-target edits. For these reasons, HDR is much less exact and less widely used than NHEJ.
Nonhomologous End-Joining (NHEJ)
Opposed to HDR, the use of targeted nucleases through NHEJ have been found as an alternative approach to increase the efficiency of genomic editing. In NHEJ, DSBs do not require a homologous template to guide repair, eliminating the need for HR. NHEJ instead creates two targeted DSBs, located on both strands of the cellular DNA, to totally delete an identified target sequence from the genome. In this way, NHEJ gene correction can be used as a therapeutic deletion of a mutated sequence.
NHEJ-mediated repair has many advantages over HDR because methods are generally simpler, there is no need for a repair matrix, and that NHEJ may be active through the entirety of the cell cycle and not in specific phases. NHEJ may also be applied in immortalized cell lines to inactivate a single gene or multiple genes through creating loss-of-function mutations. This, in turn, can lead to permanent gene inactivation.
NHEJ may result in errors as the process intrinsically forms gene insertions and/or deletions (termed indels) in diverse lengths at the DSB site. If indels occur in the coding sequence frameshift mutations may result which could lead to mRNA degradation, nonfunctional truncated protein production by nonsense-mediated decay, or the inactivation of an entire gene.
Laboratory Techniques
Zinc-finger nucleases (ZFNs)
Zinc-fingers are segments of proteins that have evolved to recognize and bind to specific DNA sequences. A ZFN system exploits this function, created in the 1990s to improve the specificity of genome editing and reduce off-target edits. A ZFN system firstly requires the generation of artificial proteins that consist of a customizable sequence-specific DNA-binding domain. Each of these artificial proteins is then connected to a nonspecific nuclease for highly targeted cleavage. After cleavage, the cut DNA sequence may be deleted or replaced with a new one via HR.
Though ZFNs have improved the success rate of HR genome editing by roughly 10% over traditional methods of HR, the process is lengthy, steps are time-consuming, and pose difficulty in design, construction, and the production of zinc-finger proteins. Each ZFN is tailored for every targeted DNA sequence, so new ZFNs must be engineered for each desired distinct genome edit.
Datasets: | |
Transcription activator-like effector nucleases (TALENs)
Other possibilities for more precise genome editing have more recently derived from a certain class of flavobacteria termed transcription activator-like effectors (TALEs). TALEs are secreted as bacterial proteins with DNA-binding domains that contain a series of conserved sequences, 32-34 residues in length, containing two divergent amino acids. These divergent amino acids are largely responsible for determining the DNA-binding specificity of the construct.
Like ZFNs, TALENs are engineered from proteins found in nature and are capable of binding to specific DNA sequences. TALENS contain a DNA-binding protein that not only recognizes, but fuses, and cleaves these particular DNA sequences. Although the applications of ZFNs and TALENs are very much overlapping in how efficiently they can edit a genome, TALENs offer greater simplicity in design and construct, due to a robust recognition code. Though TALENs can cleave any DNA sequence of interest with relatively high frequency, the main challenges in this approach lies in that they require complex molecular cloning for each new DNA target, and are lowly efficient at genome screening in targeted cells.
Meganucleases (MegNs)
MegNs are naturally occurring endodeoxyribonucleases, molecules that are both endonucleases and deoxyribonucleases that catalyze cleavage of the phosphodiester bonds in DNA.
MegNs are found within all forms of microbial life, and are also seen in eukaryotic mitochondria and chloroplasts. MegNs contain long DNA-binding recognition sites, generally up to 40 nucleotides, and genes that encode MegNs are often embedded within self-splicing locations. Though MegNs are more specific than other genetic editing tools for the development of therapies, due to their length it is often unlikely that natural genomic editing sites are present, especially in the human genomes.
Applications of MegNs in genome editing are limited and technically challenging. MegNs are difficult to design, engineer, and processes are time-consuming and expensive. In spite of so many available MegNs, they are unlikely to be used outside of research regularly for genome editing given the relative simplicity of the alternative methods.
Datasets: | |
Clustered Regularly Interspaced Short Palindromic Repeat (CRISPR)
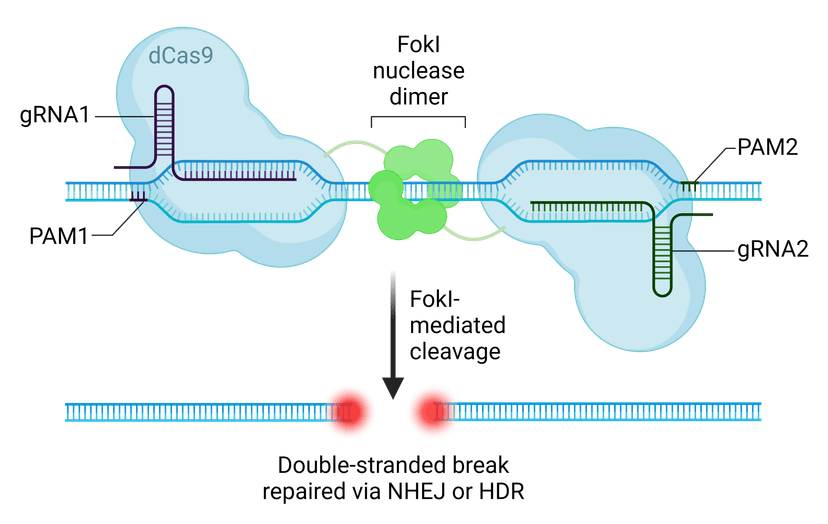
Simplified process of CRISPR/Cas9 gene editing complex. The guide RNA strands permit targeting gene editing resulting in a broken strand that can then be processed further via NHEJ or HDR. Figure made in Biorender.
The CRISPR/Cas9 complex works with two small RNAs, CRISPR RNA (crRNA) transcribed from the CRISPR locus, and a trans-activating crRNA (tracrRNA). crRNA and tracrRNA then form a chimera, guide RNA (gRNA), that programs Cas9 to cleave a targeted DNA site.
Unlike other methods, CRISPR offers a simple technology with minimal assembly and experimental steps. In simplified terms:
- First, a short RNA template must be created that matches a target DNA sequence in the genome, which is much easier than engineering the alternative nuclease systems mentioned previously.
- Next, complementary strands of RNA and targeted DNA bind and gRNA then directs Cas9 to cut and/or edit the genome at a targeted location.
Note: CRISPR can engineer deletions as well as insertion in the genome, and some research has shown that this technique is 600% more effective than TALENs in creating targeted genomic edits. CRISPR has allowed large-scale genomics projects, which once took years and thousands of dollars, to be accomplished for a fraction of time and cost.
The versatility of CRISPR technology has been rapidly expanding from genomic sequence corrections and/or alterations into epigenetic and transcriptional modifications. It is frequently used in combination with HDR and NHEJ for gene knock-in and knockout, respectively. Though novel, CRISPR does come with some downsides in that it exhibits a lack of on-target editing leading to the major potential for off-target effects, and that there exists a high potential for incomplete editing (mosaicism).
Product Ordering Information
Table 1. DNA Sequencing Building Blocks
Cat# ▲ ▼ | Product Name ▲ ▼ | Unit Size ▲ ▼ |
300 | 5-dR6G [5-Carboxy-4,7-dichlororhodamine 6G] | 25 mg |
301 | 6-dR6G [6-Carboxy-4,7-dichlororhodamine 6G] | 25 mg |
302 | 5-dR6G, succinimidyl ester | 5 mg |
305 | 5-dTMR [5-Carboxy-4,7-dichlorortetramethylrhodamine] | 25 mg |
306 | 6-dTMR [6-Carboxy-4,7-dichlorortetramethylrhodamine] | 25 mg |
307 | 5-dTMR, succinimidyl ester | 5 mg |
310 | 5-dROX [5-Carboxy-4,7-dichloror-X-hodamine] | 25 mg |
311 | 6-dROX [6-Carboxy-4,7-dichloror-X-hodamine] | 25 mg |
312 | 5-dROX, succinimidyl ester | 5 mg |
315 | 5-dR110 [5-Carboxy-4,7-dichlororhodamine 110] | 25 mg |
Table 2. Double-stranded DNA-binding dyes for qPCR
Product ▲ ▼ | Ex (nm) ▲ ▼ | Em (nm) ▲ ▼ | Unit Size ▲ ▼ | Cat No. ▲ ▼ |
Helixyte™ Green *20X Aqueous PCR Solution* | 498 nm | 522 nm | 5x1 mL | 17591 |
Helixyte™ Green *10,000X Aqueous PCR Solution* | 498 nm | 522 nm | 1 mL | 17592 |
Helixyte™ Green dsDNA Quantifying Reagent *200X DMSO Solution* | 490 nm | 525 nm | 1 mL | 17597 |
Helixyte™ Green dsDNA Quantifying Reagent *200X DMSO Solution* | 490 nm | 525 nm | 10 mL | 17598 |
Q4ever™ Green *1250X DMSO Solution* | 503 nm | 527 nm | 100 µL | 17608 |
Q4ever™ Green *1250X DMSO Solution* | 503 nm | 527 nm | 2 mL | 17609 |
Table 3. Available RNA quantifying reagents and kits.
Product Name ▲ ▼ | Ex (nm) ▲ ▼ | Em (nm) ▲ ▼ | Unit Size ▲ ▼ | Cat No. ▲ ▼ |
StrandBrite™ Green Fluorimetric RNA Quantitation Kit | 490 | 545 | 100 Tests | 17656 |
StrandBrite™ Green Fluorimetric RNA Quantitation Kit *High Selectivity* | 490 | 540 | 100 Tests | 17657 |
StrandBrite™ Green Fluorimetric RNA Quantitation Kit *Optimized for Microplate Readers* | 490 | 545 | 1000 Tests | 17655 |
StrandBrite™ Green RNA Quantifying Reagent *200X DMSO Solution* | 490 | 525 | 1 mL | 17610 |
StrandBrite™ Green RNA Quantifying Reagent *200X DMSO Solution* | 490 | 525 | 10 mL | 17611 |
Portelite™ Fluorimetric RNA Quantitation Kit *Optimized for Cytocite™ and Qubit™ Fluorometers* | 490 | 525 | 100 Tests | 17658 |
Portelite™ Fluorimetric RNA Quantitation Kit *Optimized for Cytocite™ and Qubit™ Fluorometers* | 490 | 525 | 500 Tests | 17659 |
Cell Navigator® Live Cell RNA Imaging Kit *Optimized for Fluorescence Microscope* | 490 | 520 | 100 Tests | 22630 |
References
Gene Editing - Digital Media Kit
Applications of genome editing technology in the targeted therapy of human diseases: mechanisms, advances and prospects
The Basic Science of Genome Editing
How Does Genome Editing Work?
Homology-directed repair (HDR) with a plasmid donor
The genome editing revolution: review